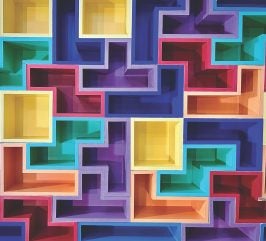
A team of MIT and Lawrence Berkeley National Laboratory (LBNL) scientists, led by Ryotaro Okabe, have developed a computational model for designing simple and streamlined radiation sensor setups that can identify the direction of a source of radiation. By moving the sensor around to get multiple readings, the technology is capable of providing cross-referenced bearings to give a precise location.
While radiation detectors have already been developed to identify the position and strength of a radioactive source, the complex mechanisms of radiation-matter interaction have made high-performance and low-cost radiation mapping challenging to date.
Radiation is usually detected using semiconductor materials like cadmium zinc telluride (CZT), which produce an electric current when struck by radiation such as gamma rays. However, because radiation easily penetrates through matter, it’s difficult to determine the direction that such a signal emerges from. The large penetration depth of radiation reduces the angular sensitivity of detectors and limits the majority of radiation detection efforts to focus on counting or spectra acquisition rather than their directional information. They cite Geiger counters as an example, which simply provides a click when receiving radiation, without providing information on the energy or type of radiation. Finding a source thus requires moving the detector to locate the maximum intensity. The process therefore requires the user to move closer to the source of radiation, potentially increasing the exposure risk.
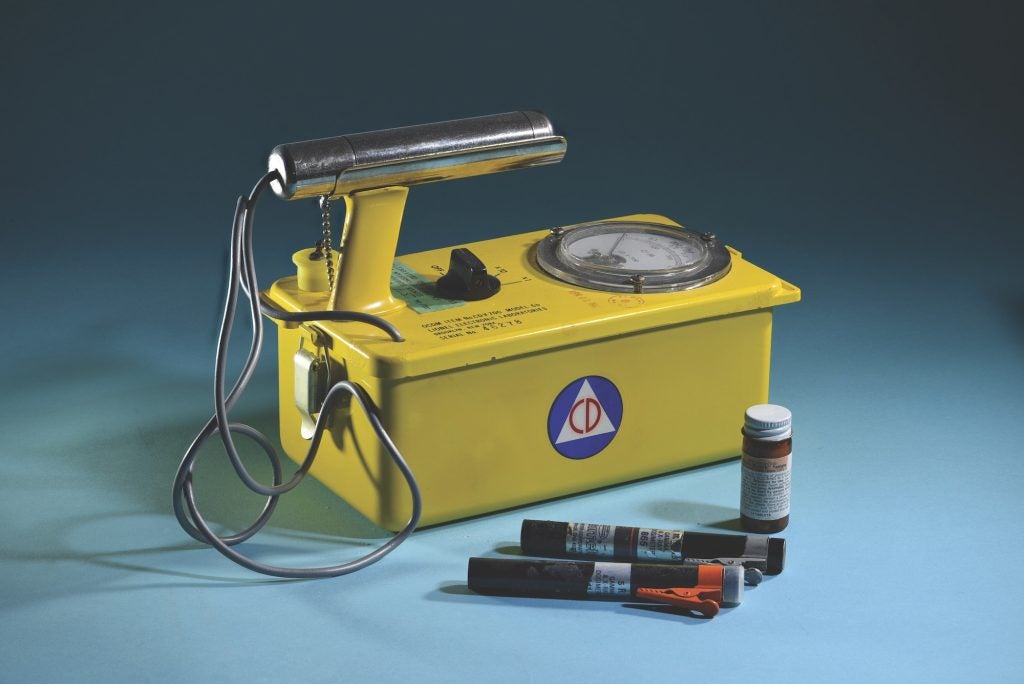
The challenge of acquiring directional radiation information further triggers additional difficulties in performing source localisation and to determine the position of radiation sources. To provide directional information from a stationary device without getting too close, researchers use an array of detector grids along with a mask. This mask produces a different pattern on the array depending on the direction of the source. For example, the High-Efficiency Multimode Imager (HEMI) consists of two layers of CZT detectors. The first has a randomly arranged aperture while the second layer is a conventional co-planar detector grid. This system requires the incident beam to only come from a limited range of angles to pass through the aperture of the first layer and to interact with the second layer. An algorithm interprets the different timings and intensities of the signals received by each individual detector or pixel to provide a direction but this approach often leads to complex detector design. Typically, such detector arrays capable of sensing the direction of a radiation source are large and expensive with a 10 by 10 array and at least 100 pixels. Not only are the individual detector elements expensive, but all of the interconnections carrying information from those pixels also become much more complex.
The traditional reconstruction algorithm also requires all the incident beams to come within the field of view. Accuracy will be affected if the radiation is incident from another direction (especially for near-field radiation). In addition, this type of system can only conditionally detect multiple sources, usually when the sources come from different isotopes and can be distinguished by energy. In this scenario, the detection with multiple sources can be reduced to single-source detection by only considering the count of events within an energy range. However, in real-world applications, different sources are not necessarily distinguishable in the energy spectrum.
Another approach uses detectors separated by padding shielding material. Radiation sources from different directions and distances can result in different intensity distribution patterns over detector arrays. However, because of the inaccuracy of the model caused by misalignment and manufacturing errors of detector and shielding material, it is challenging to extract information from detector data via these traditional methods.
Thus while there have been versions of simplified arrays for radiation detection, many are only effective if the radiation is coming from a single localised source and they can be confused by multiple sources or sources that are spread out.
An alternative approach to detection
As an alternative, the combined MIT/LBNL team developed a radiation mapping framework using detector pixels that have been inspired by the video game Tetris. According to a recent paper from the team and published by Nature Communications, Okabe and MIT professors Mingda Li, and Benoit Forget, senior research scientist Lin-Wen Hu, and principal research scientist Gordon Kohse; graduate student Shangjie Xue; research scientist Jayson Vavrek at LBNL, and a number of others at both institutions, found that using as few as four pixels arranged in the tetromino shapes of the figures in the “Tetris” game can come close to matching the accuracy of the large, expensive systems. Shapes from the Tetrominoes family are composed of four squares. The author’s radiation detection framework uses a minimal number of detectors, combining Tetris-shaped detectors with inter-pixel padding, along with a deep-neural-network-based detector reading analysis. The inter-pixel padding material is included to intentionally increase contrast.
One of the major breakthroughs to make the system work is placing an insulating material between the pixels to increase the contrast between radiation readings coming into the detector from different directions. The contrast between pixels is thus not only created by incident angles but enhanced by padding layers that are good absorption layers of radiation. The inter-pixel padding material is chosen to be 1 mm-thick lead, which is thick enough to create contrast and with quite a low photon absorption in the γ-ray range. The lead serves the same function as the more elaborate shadow masks used in the larger conventional systems.
The detector pixels are composed of CZT with a size of 1 cm2 each, slightly larger than current crystals used in detectors but still much smaller than the 5 metre source to detector distance. The detector grid is placed horizontally within the plane instead of vertically facing the source.
The key to this method of detection is proper computerised reconstruction of the angles of arrival of the rays, based on the times each sensor detects the signal and the relative intensity each one detects, as reconstructed through an AI-guided study of simulated systems. Machine learning algorithms are implemented to analyse the detector reading, demonstrating great promise to reduce the need for detector pixel numbers and thereby reduce the cost of fabrication and deployment.
Different configurations of four pixels were tried by the researchers with an evaluation of the prediction accuracy of detectors which comprise with following four detector configurations: 2 × 2 square grid, and Tetrominos of S-, J-, T-shapes. An I-shaped Tetris detector array is not presented since it does not show performance good enough for directional mapping.
Less symmetrical arrangements, the team found, provide more useful information from a small array. While the S-shape detector worked with the smallest prediction followed by 2 × 2 square, J- and T-shapes. Although the T-shaped Tetris is the least accurate, all of the four types of detector could work enough to know the direction of the radiation source with about 1° of accuracy.
Throughout the study, the authors assume that the incident beam energy is γ-radiation of 0.5 MeV, which they suggest is the realistic energy from pair production and comparable to many γ-decay energy levels.
Advancing detector AI
Using neural networks trained with Monte Carlo (MC) simulation data, a detector with as few as four pixels can achieve high-resolution directional prediction and shows this approach can potentially have a higher resolution than more complex alternatives. The authors suggest that experimental validation could further prove the capability of machine learning approach for locating radiation sources in the real-world scenario.
In real-world applications of radiation mapping, the authors note that it would be highly desirable to go beyond directional information and determine the exact position of a radiation source. They propose a method based on Maximum A Posteriori (MAP) estimation generate the distribution of radiation through the motion of the simple detector. The detector readout is simulated by MC given the detector’s initial position and orientation before the detector begins to move in a circular motion. During the detector motion, the predicted source direction is calculated based on the model before the location of the radiation source is estimated via MAP based on the series of neural-network-inferred detector direction data at different detector positions. In an ideal case for one single radiation source, as few as two positions are enough to locate the source position while the circular motion and MAP are implemented for more complicated radiation profile mapping.
Using a moving detector with MAP thus took this development further and succeeded in localising the position of a radiation source. Field testing with a simple detector in a single-blind field test at the Berkeley Lab with a real caesium radiation source subsequently verified the capability of the MAP method for localisation of radiation sources with high accuracy in determining both the direction and distance to the source. This element of the research was led by Vavrek, in which the researchers at MIT did not know the location of the radiation source.
In their paper, the authors note incidents like the Fukushima Daiichi Nuclear Power Plant disaster in Japan in 2011 and the threat of a possible radiation release from Zaporizhzhia in the Ukrainian war zone underscoring the need for effective detection and monitoring of radioactive isotopes. The machine learning-based algorithm and aerial radiation detection will allow real-time monitoring and integrated emergency planning of radiological accidents for example.
However, the authors also note that everyday operations of nuclear reactors, uranium mining and processing, and the disposal of spent nuclear fuel also require monitoring of radioisotope release. In recent years, radiation localisation has also attracted increased interest with applications such as autonomous nuclear site inspection.
In this study they authors focused on gamma-ray sources but the computational tools they developed to extract directional information from the limited number of pixels aren’t restricted to particular wavelengths and can therefore also be used for neutrons, or even other forms of light, such as ultraviolet. The authors conclude that their framework offers an avenue for high-quality radiation mapping with simple detector configurations and is anticipated to be deployed for real-world radiation detection.